The Physics of Fragmentation
Liquid jets: Fluid motion around a bubble can lead to a high speed liquid jet that forms during the collapse of a bubble. Figure 1a shows a high-speed video sequence of such a bubble. The jet that’s formed can be very powerful. It acts as a water hammer and can damage even hard objects. Damage to boat propellers are linked to this mechanism. Imagine that a small bubble can damage a hard metal, now that’s impressive! The jet velocity can be hundreds of meters/second, leading to impact pressures of hundreds of MPa. Figure 1b shows a closeup of the jet and a video of the dynamics.
Figure 1a. Bubble collapse leading to a fluid jet pointed downwards. Citation: Werner Lauterborn and Claus-Dieter Ohl,
“The Peculiar Dynamics of Cavitation Bubbles,” Applied Scientific Research, Vol. 58, pp 63–76 (1998).
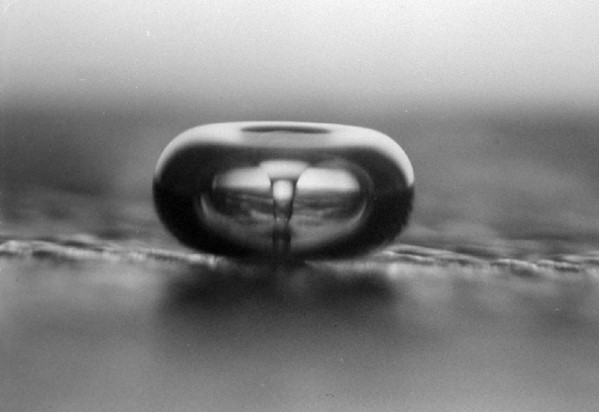
Figure 1b. Liquid jet through a bubble. Image and movie courtesy of Lawrence Crum, Ph.D.
Figure 2. Shock wave emission from a spherical bubble collapse. Video courtesy of Thomas J. Matula, Ph.D.
Microstreaming: Cavitation (i.e., oscillating bubbles) induces microstreaming which generates large shear stresses on nearby objects, including DNA. This phenomenon is the most important mechanism for DNA fragmentation. An example image and movie is shown in figure 4.
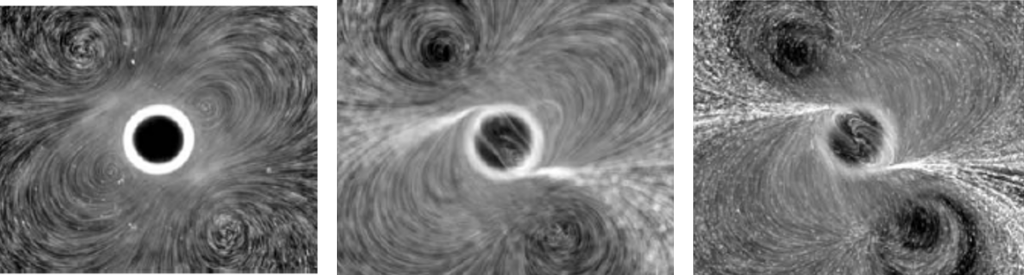
Figure 3. Shear stresses induced on particles near an oscillating bubble. (a) Citation: Paul Tho, Richard Mannasseh, and Andrew Ooi, “Cavitation microstreaming patterns in single and multiple bubble systems,” Journal of Fluid Mechanics; Vol. 576, pp 191-233 (2007). (b) Video courtesy of Richard Mannasseh, Ph.D.
Sonoluminescence: What’s it like inside a bubble that collapses violently? This was one of the hottest physics topics in the 1990s, because controlling the bubble’s collapse led to it emitting a flash of light, which was termed “sono-luminescence,” or “light from sound.” Essentially, the final stage of the bubble’s collapse is nearly adiabatic. The temperature at the bubble’s center can get hotter than the surface of the sun, with corresponding dissociation of molecular bonds, ionization and radical formation. Figure 3a shows the dynamics of how a bubble can grow and collapse symmetrically to generate a sonoluminescence flash. Figure 3b shows the light emission. While this is impressive, in reality the heat and light come from the very center of the bubble, while near the surface of the bubble it’s already back to ambient conditions. The light flash itself is very short (often measured in picoseconds), but under controlled conditions, it can flash every acoustic cycle, tens of thousands of times per second, making it look like it’s continually emitting. While this mechanism is certainly possible in sample preparation, it requires near perfect symmetrical collapse, which doesn’t happen much during processing. Still, the phenomenon is so cool, we just had to include it.
Figure 4. Sonoluminescence bubble. (a) Bubble dynamics. Citations: Thomas J. Matula, “Inertial Cavitation and sonoluminescence,” Phil. Trans. R. Soc. Lond., 357, 225-249 (1999). Thomas J. Matula, “Single-bubble sonoluminescence in microgravity,” Ultrasonics 38, 559-565 (2000). (b) Standard video of light emission. Video courtesy of Thomas J. Matula, Ph.D.
Frequency effects: The mechanisms described above can occur at frequencies ranging from 20 kHz (or lower) all the way up into the MHz range. All sonication instruments generate cavitation. The most basic lab instrument is the ultrasonic horn (also called a “cell disruptor,” “homogenizer,” “Sonotrode,” etc.). It operates at the low end of the frequency spectrum, around 20-40 kHz or so. The low end is determined by the desire to stay above the threshold for hearing. Hence, you won’t see any instruments working below 20 kHz. Even those operating at 20-40 kHz can generate a significant amount of audible noise, because of vibrations induced in other components. The high end of operation is limited by the ability of cavitation to do work. When a bubble grows, it absorbs potential energy, and that energy is converted into kinetic energy during collapse, which is then used to do work. Making a bubble grow for a longer period of time (=low frequency) would impart more energy into the system, while making a bubble oscillate more frequently (= high frequency) allows the bubble to do more work per unit time. Another big tradeoff is that lower frequency devices are larger because the transducers are bigger. Going up in frequency allows you to reduce the footprint of your instrument.
Sample Heating: All bubbles absorb energy from the acoustic wave, and some of that energy is dissipated as heat. That’s the physics, and there’s no getting around it. Heating is controlled by cooling the samples and optimizing acoustic pulse parameters.